Dosimetric study of Qfix kVue rails for pencil beam scanning proton beam
Introduction
Patient support structures are known to have dosimetric effects that are not negligible. AAPM TG-176 (1) summarizes the dosimetric impact of couch tops for both photon and proton radiation, and provides extensive guidelines on commissioning measurement requirements as well as avoidance strategies in the clinic. Subsequent works compared multiple proton couch tops together with accessory immobilization devices (2,3). These studies provided general guidelines on proper beam angle arrangements to minimize the dosimetric interference from couch tops. However, certain clinical conditions, such as re-treatment or extensive lesion size in the medial-posterior section, could limit the available beam angles to the planners and optimal beam avoidance to the rails or table edge may not be achievable for those cases. Such situations could potentially push the beam very close to the unfavorable table structures, e.g., the underlying rails and/or uneven table edges. Slight misalignment of the patient relative to the table top, in this case, could have a large dosimetric effect that is not captured by the treatment planning system (TPS). In this study we examined and quantified the dosimetric effects of unfavorable couch top structures such as rails.
The kVue system is widely used in proton beam therapy (4). It is composed of a fixed base and an interchangeable tabletop. With a physical thickness of 2.8 cm and a water equivalent distance of about 0.5 cm, the tabletop is composed of low density carbon fiber and has low attenuation under both photon and proton radiation, and instigates minimal artifacts with cone beam computed tomography (CT). The wide variety of interchangeable tabletops that can all fit into the same base made the kVue system one versatile patient support device for patient treatment. However, in order to support the weight of the patient, two rails composed of higher density carbon fiber must be placed under the thin table top (Figure 1). The rails are attached to the base which, in turn, is fixated to the robotic arm that is capable of bearing a weight of 500 pounds.
During simulation, the removable tabletop is used and included into the patient’s CT scan for dose calculation. The rails, however, are not used and therefore not included in the patients’ CT scan. This discrepancy between the CT scan and the actual treatment table must be carefully managed in order to ensure correct dose calculations. To reconcile this discrepancy, outlines of the rails are inserted into the patient’s structure set to assist the planners in visualizing the position of the rails. Beam angles are then selected such that no beams will go through the rails. Note that the rails are movable under the base of the kVue system, i.e., the left and the right rails do have a limited range where they can be either pushed inward to the center of the tabletop, or outward to the edge of the tabletop. This ability to configure the position of the rails, together with the use index bars to shift the patient laterally on the table, allows the designed treatment plans to have all proton beams avoid traversing the rails. However, for actual treatments, the position of the patient may not always be centered at the table, or at the designed location of the index bar (in the case that a lateral index bar is used). In practice, we notice that the position of the patient relative to the tabletop can vary up to 2 cm from day to day, as evidenced by the allowable ranges of the tabletop positions in weekly chart checks. Inevitably, there are cases where the edges of the beam can skim very closely to the rails. Depending on the exact beam angles and the angled structures within the rails, deviation of the actual dose distribution from that seen in the TPS can become significant. In this study, we quantitatively examine the dosimetric effects of the rails using both a water tank and a patient CT. We present the following article in accordance with the MDAR reporting checklist (available at https://tro.amegroups.com/article/view/10.21037/tro-22-9/rc).
Methods
In this study, the kVue tabletop with rails was placed on a simulation table and scanned using a GE Optima CT580, with X-ray tube voltage at 120 kV and slice thickness of 2.5 mm. The test proton plans were made using Varian’s Eclipse TPS (Varian Medical Systems, Palo Alto, CA, USA) for a water tank and a patient.
For the study with a water tank, a box contour was drawn on top of the kVue couch with rails, and its Housfield unit (HU) values were assigned to be water. It is more than 50 cm × 50 cm in cross section and about 22 cm in height. Test plans were generated at various gantry angles using the pencil beam scanning technique (5-7), targeting a cuboid clinical target volume (CTV) with 5 mm margin. The HU of the table top and the rails were assigned to be air in the plan optimization, emulating a typical clinical plan where the beams avoid the rails. After the optimization is completed, the HU assignment to air was removed so that the tabletop and the rails’ actual HUs were used to evaluate their dosimetric impact. The kVue tabletop is designed to have a thin exterior layer with a low density core that combine to give a near constant water equivalent distance within a single beam regardless of the direction of the incident beam angle. The water equivalent thickness is about 0.5 cm for vertically incident beams based on our Eclipse TPS. This is consistent with the values reported in earlier studies using the same model couch top (2,3). Unlike the flat and relatively uniform table top, the rails on the other hand have internal structures that can induce different water equivalence distances depending on the beam’s incident angle and locations.
For the study with a patient scan, the proton plan was optimized on an anonymized patient scan with a tabletop, but without the rails. After the optimization is completed, the plan was copied to another CT scan with rails contoured and HU overridden beneath the table. An in-house Monte Carlo dose engine based on MCSquare was used to calculate the dose distributions (8-10). The dose distributions from both plans was compared to evaluate the effect of rails.
Results
Case A: posterior-anterior (PA) beam on a water phantom
Figure 2 shows the dose distributions of a cuboid, optimized to have uniform dose distribution without the rails. The nominal energy is 154 MeV with 16 cm range, 11 cm spread-out Bragg peak (SOBP) and 20 layers. The same plan was then re-calculated with the rails inserted beneath the table. The water equivalent distance and dosimetric effect of the rails was evaluated by comparing the original uniform dose distribution and the dose distributions perturbed by the rails (Figure 3). The water equivalent distance of the rails varies from 0.6 to 3.8 cm, depending on the relative orientation of the rails’ internal structures and the beam angle used. Note that the largest perturbation is on the outer edge where the beam traverses parallel to an angled frame. The impact on the dose varies at different locations accordingly, with the biggest impact again on the outer edge. Figure 4 shows an example Gamma test (11) between doses with and without rails in the beam path.
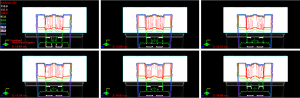
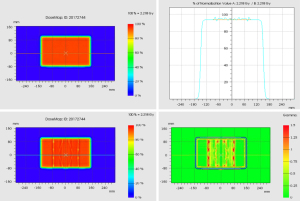
Case B: posterior oblique beams on a water phantom
For an oblique beam as shown in Figure 5, the kVue tabletop has a slightly longer water equivalent distance than the PA beam, and pulls back the isodose lines slightly more than the PA beam. The nominal energy for this right posterior oblique (RPO) beam is 159 MeV with 17 cm range, 11 cm SOBP and 20 layers. As shown in Figure 6, the water equivalence distance of the rails varies at different locations, with the largest on a left rail’s inner edge which is almost parallel to the beam path.
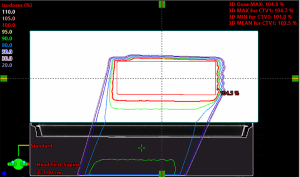
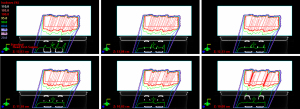
Case C: disturbance of dose on a test patient
A test patient plan was optimized with the tabletop in the CT but no rails (Figure 7, left column). The target volume is the lumbar vertebrae. For the RPO beam in the patient plan, the nominal energy is 183 MeV with 22 cm range, 13 cm SOBP and 21 layers. The nominal energy for the LPO beam is 175 MeV with 20 cm range, 13 cm SOBP and 22 layers. The test plan was then copied to the scan with the rails contours in the beam path (Figure 7, right column). The HU of the rails contours are assigned to be 200 based on the proton stopping power of the physical rails. The doses between those two plans were compared at various locations. The gamma analysis was also done between these two plans (Figure 8). The beam’s eye view, as seen from the two posterior oblique beams, is shown together with the rail structures and the proton spots (Figure 9).
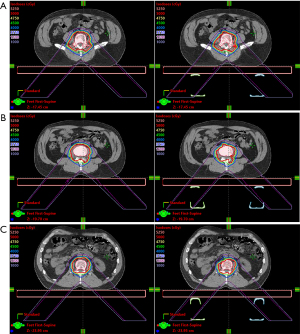
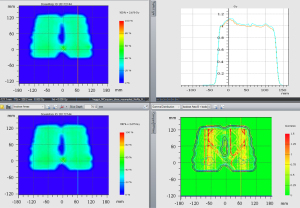
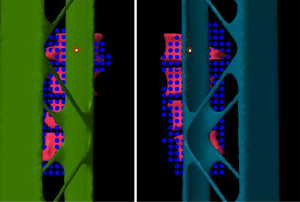
Conclusions
The kVue system’s rails do have a dosimetric impact on the dose distribution that is not negligible. As seen in this study, the rails should not be in the beam path for treatment for plans that are optimized without specifically taking the rails into consideration. For treatment plans with posterior beams, it is therefore important for the therapy staff to ensure the rails are positioned at the designated location. Currently, newer tabletops that do not require weight supporting rails are also being developed to simplify the treatment process.
Acknowledgments
The authors thank the California Protons Cancer Therapy Center for the clinical data support.
Funding: None.
Footnote
Provenance and Peer Review: This article was commissioned by the editorial office, Therapeutic Radiology and Oncology, for the series “Pencil Beam Scanning Particle Therapy”. The article has undergone external peer review.
Reporting Checklist: The authors have completed the MDAR reporting checklist. Available at https://tro.amegroups.com/article/view/10.21037/tro-22-9/rc
Data Sharing Statement: Available at https://tro.amegroups.com/article/view/10.21037/tro-22-9/dss
Conflicts of Interest: All authors have completed the ICMJE uniform disclosure form (Available at https://tro.amegroups.com/article/view/10.21037/tro-22-9/coif). The series “Pencil Beam Scanning Particle Therapy” was commissioned by the editorial office without any funding or sponsorship. CC served as the unpaid Guest Editor of the series. The authors have no other conflicts of interest to declare.
Ethical Statement: The authors are accountable for all aspects of the work in ensuring that questions related to the accuracy or integrity of any part of the work are appropriately investigated and resolved.
Open Access Statement: This is an Open Access article distributed in accordance with the Creative Commons Attribution-NonCommercial-NoDerivs 4.0 International License (CC BY-NC-ND 4.0), which permits the non-commercial replication and distribution of the article with the strict proviso that no changes or edits are made and the original work is properly cited (including links to both the formal publication through the relevant DOI and the license). See: https://creativecommons.org/licenses/by-nc-nd/4.0/.
References
- Olch AJ, Gerig L, Li H, et al. Dosimetric effects caused by couch tops and immobilization devices: report of AAPM Task Group 176. Med Phys 2014;41:061501. [Crossref] [PubMed]
- Wroe AJ, Ghebremedhin A, Gordon IR, et al. Water equivalent thickness analysis of immobilization devices for clinical implementation in proton therapy. Technol Cancer Res Treat 2014;13:415-20. [PubMed]
- Wroe AJ, Bush DA, Slater JD. Immobilization considerations for proton radiation therapy. Technol Cancer Res Treat 2014;13:217-26. [Crossref] [PubMed]
- Paganetti H, Beltran C, Both S, et al. Roadmap: proton therapy physics and biology. Phys Med Biol 2021; [Crossref] [PubMed]
- Lomax AJ, Goitein M, Adams J. Intensity modulation in radiotherapy: photons versus protons in the paranasal sinus. Radiother Oncol 2003;66:11-8. [Crossref] [PubMed]
- Unkelbach J, Paganetti H. Robust Proton Treatment Planning: Physical and Biological Optimization. Semin Radiat Oncol 2018;28:88-96. [Crossref] [PubMed]
- Frank SJ, Cox JD, Gillin M, et al. Multifield optimization intensity modulated proton therapy for head and neck tumors: a translation to practice. Int J Radiat Oncol Biol Phys 2014;89:846-53. [Crossref] [PubMed]
- Huang S, Kang M, Souris K, et al. Validation and clinical implementation of an accurate Monte Carlo code for pencil beam scanning proton therapy. J Appl Clin Med Phys 2018;19:558-72. [Crossref] [PubMed]
- Souris K, Lee JA, Sterpin E. Fast multipurpose Monte Carlo simulation for proton therapy using multi- and many-core CPU architectures. Med Phys 2016;43:1700. [Crossref] [PubMed]
- Huang S, Souris K, Li S, et al. Validation and application of a fast Monte Carlo algorithm for assessing the clinical impact of approximations in analytical dose calculations for pencil beam scanning proton therapy. Med Phys 2018;45:5631-42. [Crossref] [PubMed]
- Chang C, Poole KL, Teran AV, et al. Three-dimensional gamma criterion for patient-specific quality assurance of spot scanning proton beams. J Appl Clin Med Phys 2015;16:381-8. [Crossref] [PubMed]
Cite this article as: Qu L, Liu L, Giebeler A, Chang C. Dosimetric study of Qfix kVue rails for pencil beam scanning proton beam. Ther Radiol Oncol 2022;6:15.